Terminology note: I will use the term “peptide” to refer to chains of amino acids that are short (50-100 amino acids) relative to the size of a typical protein (300-500 AA).
Proteins as Nanotechnology
Proteins perform virtually all biological functions and are responsible for synthesizing every structure in your body. The fact that these biomolecules can be used to create motors, perform chemical synthesis, construct macroscale structures, and manipulate light suggests that they may be a good basis for nanotechnology.
Problem: The high cost of producing large proteins limits their application to highly-profitable domains such as medicine. The costs and time required for protein production makes it difficult to iterate, making protein design extremely challenging. Typically, researchers modify or repurpose proteins found in nature and are limited to existing biological precedent. Reducing costs and streamlining the design process is critical to unlocking new applications.
Solution: Break the protein production and design process into smaller pieces by separately designing peptides for specific roles. These peptides are far cheaper and faster to produce, accelerating the design cycle. Multiple peptides can be combined together into larger proteins with new properties.
Modular Peptides as a New Design Approach
Specialized peptides
Solid-phase synthesis lets us produce a huge array of peptides quickly and at low cost. With fast and cheap production cycles, peptides can be designed for specific roles as antibodies, drug-delivery systems, catalysts, linkers, and sensors.
To increase effective yield and lower purification costs, these peptides can be designed specifically to be robust to synthetic errors, fold consistently, and crystallize easily.
Modular proteins
Using native chemical ligation or linker peptides, we can combine several specialized peptide “modules” into a single protein to achieve new functionalities. For example, a cancer-binding module combined with a fluorescent module could stain cancer cells and help surgeons excise cancerous tissue. Alternatively, the fluorescent module could be swapped with a drug delivery system to target cancer cells directly.
A more sophisticated application could use an aptamer module to grab a particular molecule, a catalyst module to convert it into a desired product, and a “linker” module that arranges these components into a sponge-like array. This structure could be used to filter specific molecules from wastewater and produce desired compounds.
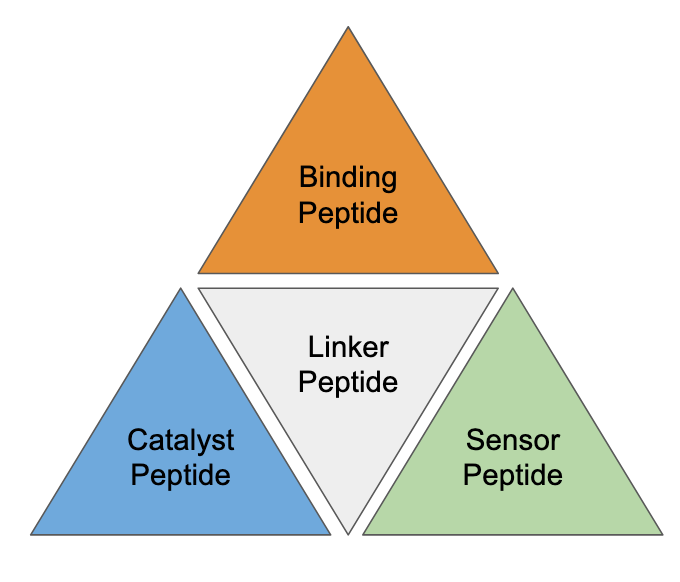
Appendix
Current vs. Theoretical Energy Efficiency of Protein Production
Let’s use broiler chickens to estimate the current energy required to produce food protein. Broiler chickens are raised for roughly 50 days and production of 100 g of protein requires 7 m2 of land (to both grow feed and raise the chickens). The energy opportunity cost of this land depends on how much solar energy could be produced from it. Assuming a solar panel produces 1 kWh/m2/day, then 100g of chicken protein costs:
50 days * 7 m2 * 1 kWh/m2/day ≈ 1.4E9 J
Let’s compare this to the energy cells require to synthesize proteins in a ribosome. The ribosome requires roughly 1 molecule of ATP per amino acid when synthesizing protein.
100 g protein is roughly 0.9 mol of amino acids (AA), requiring 0.9 mol of ATP for synthesis. Hydrolysis of ATP produces 20.5 kJ/mol. So synthesis of 100g of protein requires:
100g protein * (1 mol / 115g AA) * (1 mol ATP / mol AA) * 20.5 kJ/mol ATP ≈ 1.8E4 J
So food protein (which is cheap relative to other methods of protein synthesis) could potentially be produced with 5 orders of magnitude lower energy costs. This suggests that direct chemical methods such as solid-phase synthesis could significantly lower resource inputs required to produce designer proteins relative to farming or fermentation.